Innovative Software for
Telecom and Cybersecurity
Our cloud-native software connects, optimizes, and secures services for mobile and fixed subscribers, enterprises, and the Internet of Things. More than 100 communication service providers and 4.5 billion people rely on Enea technologies daily.
Events & Webinars
RSA Conference 2024, San Francisco
Read moreOur Solutions
Recent Awards & Recognitions
Innovation is deeply ingrained in the core of our operations. These are some of our most recent Industry awards and recognitions.
Sustainability at Enea
At Enea, we deliver on our commitments to environmental sustainability, social engagement and corporate governance through the impact of our products, and how we conduct our operations.
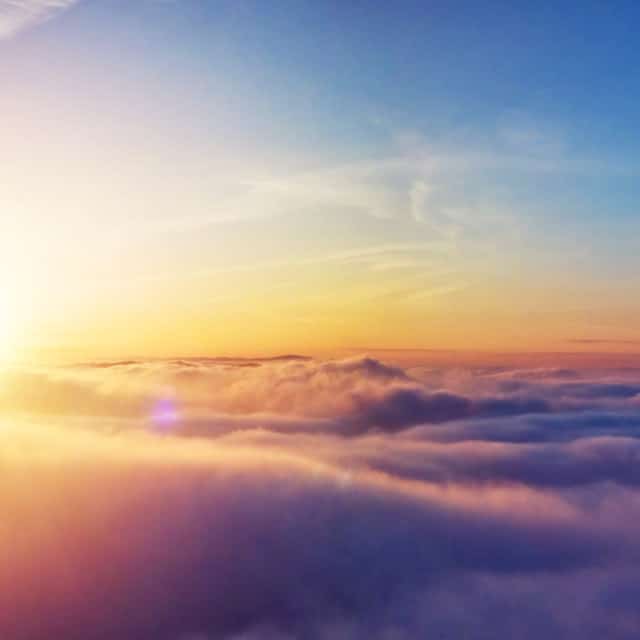
Working at Enea
Are you our next colleague?
We believe that great teamwork is the foundation for innovation. And, at Enea, there is plenty of room for talent to develop.
Welcome to our growing team!
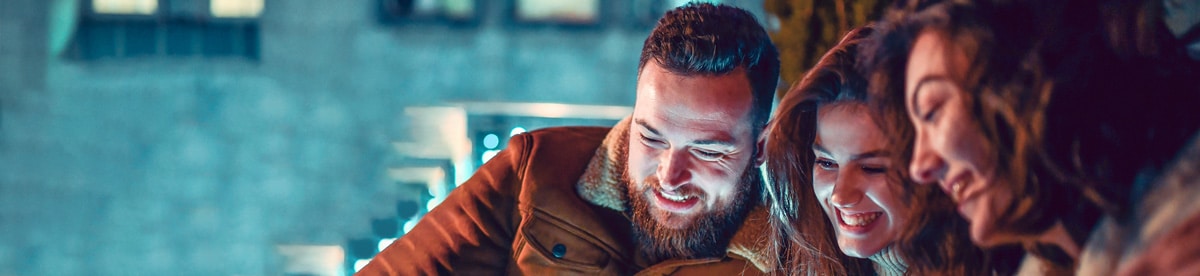
Enea AdaptiveMobile
Security has moved in!
We want to provide a complete overview of our offering. The website for Enea AdaptiveMobile Security has therefore moved to Enea’s main website. You can now explore our whole telecom and cybersecurity portfolio under Our Solutions and Your Business, and learn about trends and technology in our blogposts, podcasts, and webinars that are published under Insights.